- Author: Surendra K. Dara
- Author: Suchitra S. Dara
- Author: Alor Sahoo
- Author: Stefan Jaronski, USDA-ARS
The western Grapeleaf skeletonizer (WGLS), Harrisina metallica Stretch (Lepidoptera: Zygaenidae), previously known to cause severe defoliation to vineyards and backyard grapevines appears to be re-emerging in California. Since its first detection in San Diego in 1941, WGLS spread through commercial vineyards and backyard grapes becoming a serious problem. Although two biological control agents from Arizona and Mexico were introduced in California for WGLS control, a naturally occurring granulovirus (Harrisina brillians granulovirus) nearly eradicated WGLS populations and kept them under control. WGLS has not been a problem especially in conventional vineyards. However, based on some unpublished observations, WGLS populations are emerging in organic vineyards and backyard grapevines.
WGLS lives up to its name by skeletonizing and defoliating grape leaves. Organic vineyards are especially at risk and uncontrolled populations can destroy vineyards resulting in significant losses. Metallic bluish or greenish black moths lay barrel shaped yellowish eggs on the lower side of the leaves. There are five larval instars. Early instars are cream colored and develop black and purple bands in later stages. Pupation occurs in a whitish cocoon. Upon hatching, larvae start feeding side by side in a row on the lower side of leaf. Damage by younger larvae appears as whitish leaf area containing veins and the upper cuticle, which eventually turn brown. Older larvae skeletonize leaves leaving larger veins. Larvae may also feed on fruit leading to bunch rot. Severe damage can cause defoliation and sunburn of the exposed fruit.
Methodology
A study was conducted to evaluate the efficacy of six non-chemical control options that included formulations of spinosad, two subspecies of Bacillus thuringiensis, and a botanical insecticide/growth regulator along with two unformulated entomopathogenic fungal isolates native to California. Larvae were collected from an infested, untreated backyard grapevine and maintained in one gallon plastic tubs with screened lids on infested leaves. Fresh, untreated grape leaves from uninfested vines were provided daily for 3 days before starting the assay. For each treatment, five 4-5 instar larvae were placed on a grape leaf disc (rinsed in water and dried) in a Petri plate (100 mm dia) with a moist filter paper. Larvae were treated by spraying 1 ml of the treatment solution (containing Dyne-Amic as a surfactant at 0.125% vol/vol). Application rates for commercial formulations were determined based on label recommendations for 100 gallons of spray volume. Entomopathogenic fungal concentrations were also determined based on the label rates for similar commercial products. Treatments were replicated four times and the assay was conducted twice. Larval mortality was observed daily and dead larvae were removed and incubated separately. Fresh leaf discs were provided as needed to the remaining larvae. Actual and corrected (for control mortality) total mortality were calculated.Data were arcsine-transformed for statistical analysis and significant means were separated using Tukey's HSD test.
Results
Both cumulative daily mortality and total mortality significantly (P < 0.0001) differed among treatments. Entrust and M. anisopliae resulted in the highest mortality followed by B. bassiana, Neemix, and Agree. In general, feeding reduced or ceased in all larvae following treatment and could have contributed to a lower mortality in B. thuringiensis treatments. Entomopathogenic fungi emerged from all the cadavers from respective treatments. Microbial and botanical options provided good control of WGLS. These non-chemical alternatives can be effectively used in both organic and conventional vineyards. California isolates of B. bassiana and M. anisopliae demonstrated good control efficacy and the potential to be developed as microbial pesticides.
Acknowledgements: Thanks to the technical assistance of Alor Sahoo in carrying out these assays, and Certis and Corteva for providing the pesticide formulations.
References
Federici, B. A. and V. M. Stern. 1990. Replication and occlusion of a granulosis virus in larval and adult midgut epithelium of the western grapeleaf skeletonizer, Harrisina brillians. J. Invertebr. Pathol. 56: 401-414.
- Author: Sumanth S. R. Dara
- Author: Suchitra S. Dara
- Author: Surendra K. Dara
Charcoal rot, caused by Macrophomina phaseolina, is one of the important fungal diseases of strawberry in California. Macrophomina phaseolina is a soilborne fungus and has a wide host range, including alfalfa, cabbage, corn, pepper, and potato, some of which are cultivated in the strawberry production areas in California. The fungus infects the vascular system of the plant roots, obstructing the nutrient and water supply and ultimately resulting in stunted growth, wilting, and death of the plant. The fungus survives in the soil and infected plant debris as microsclerotia (resting structures made of hyphal bodies) and can persist for up to three years. Microslerotia germinate and penetrate the root system to initiate infection. Plants are more vulnerable to fungal infection when they are experiencing environmental (extreme weather or drought conditions) and physiological (heavy fruit bearing) stress.
Soil fumigation is the primary management option for addressing charcoal rot in strawberry. Crop rotation with broccoli can also reduce the risk of charcoal rot due to glucosinolates and isothiocyanates in broccoli crop residue that have fungicidal properties. Beneficial microorganisms such as Bacillus spp. and Trichoderma spp. are also considered, especially in organic strawberries, to antagonize M. phaseolina and other soilborne pathogens and provide some protection. The role of beneficial microbes in disease management or improving crop growth and health is gaining popularity in the recent years with the commercial availability of biofungicide, biostimulant, and soil amendment products. In a couple of recent strawberry field studies in Santa Maria, some of the beneficial microbial products improved fruit yield or crop health. These treatments can be administered by inoculating the transplants prior to planting, immediately after planting or periodically applying to the plants and or the soil. Adding beneficial microbes can help improve the soil microbiome especially after chemical or bio-fumigation and anaerobic soil disinfestation.
Similar to the benefits of traditionally used bacteria (e.g., Bacillus spp. and Pseudomonas spp.) and fungi (e.g., Glomus spp. and Trichoderma spp.), studies with entomopathogenic fungi such as Beauveria bassiana, Isaria fumosorosea, and Metarhizium spp. also demonstrated their role in improving water and nutrient absorption or antagonizing plant pathogens. The advantage of entomopathogenic fungi is that they are already used for arthropod pest management in multiple crops, including strawberry; now, there are the additional benefits of promoting crop growth and antagonizing plant pathogens. In light of some promising recent studies exploring these roles, a study was conducted using potted strawberry plants to evaluate the efficacy of two California isolates of Beauveria bassiana and Metarhizium anisopliae s.l. and their application strategies against M. phaseolina.
Methodology
About 6 week old strawberry plants (cultivar Albion) from a strawberry field at the Shafter Research Station were transplanted into 1.6-gallon pots with Miracle-Gro All Purpose Garden Soil (0.09-0.05-0.07 N-P-K) and maintained in an outdoor environment. They were regularly watered, and their health was monitored for about 5 months prior to the commencement of the study. Conidial suspensions of the California isolates of B. bassiana and M. anisopliae s.l. were applied one week before, after, or at the time of applying microsclerotia of M. phaseolina to the potting mix. The following treatments were evaluated in the study:
- Untreated control
- Soil inoculated with M. phaseolina
- Soil inoculated with B. bassiana 1 week prior to M. phaseolina inoculation
- Soil inoculated with M. anisopliae s.l. 1 week prior to M. phaseolina inoculation
- Soil inoculated with B. bassiana at the time of M. phaseolina inoculation
- Soil inoculated with M. anisopliae s.l. at the time of M. phaseolina inoculation
- Soil inoculated with B. bassiana 1 week after to M. phaseolina inoculation
- Soil inoculated with M. anisopliae s.l. 1 week after to M. phaseolina inoculation
Entomopathogenic fungi were applied as 1X1010 viable conidia in 100 ml of 0.01% Dyne-Amic (surfactant) solution distributed around the plant base. To apply M. phaseolina, 5 grams of infested cornmeal-sand inoculum containing 2,500 CFU/gram was added to four 5 cm deep holes around the base of the plant. Each treatment had six pots each planted with a single strawberry plant representing a replication. Treatments were randomly arranged within each replication. The study was repeated once a few days after the initiation of the first experiment.
Plant health was monitored starting from the first week after the M. phaseolina inoculation and continued for seven weeks. Plant health was rated on a scale of 0 to 5 where 0=dead and 5=very healthy and the rest of the ratings in between depending on the extent of wilting. Data from both experiments were combined and analyzed by ANOVA using Statistix software and significant means were separated using LSD test. The influence of entomopathogenic fungal treatments applied at different times as well as the combined effect of different applications within each fungus were compared for seven weeks. Ratings for some plants that were scorched from hot summer temperatures and died abruptly were removed from the analyses.
Results
Untreated control plants maintained good health throughout the observation period varying between the rating of 4.3 and 4.9. In general, plant health declined considerably from the 5th week after M. phaseolina inoculation. Plant health appeared to be slightly better in plants treated with entomopathogenic fungi, but there was no statistically significant difference in any except one instance. Plants treated with M. anisopliae one week prior to the application of M. phaseolina had a rating of 3.0 compared to 1.6 rating of plants inoculated with M. phaseolina alone.
When data from different treatments for each entomopathogenic fungus were compared, both B. bassiana and M. anisopliae s.l. appeared to reduce the wilting, but the plant health rating was not significantly different from the M. phaseolina treatment alone.
This is the first report of the impact of entomopathogenic fungi on M. phaseolina with some promise. Additional studies under more uniform environmental conditions and with more treatment options would shed more light on this approach of using entomopathogenic fungi against M. phaseolina. The current study evaluated single application of the entomopathogenic fungi and we plan to conduct additional studies with multiple applications.
Acknowledgements: We thank Dr. Kelly Ivors (previously at Cal Poly San Luis Obispo) for the pathogen inoculum and Dr. Stefan Jaronski, USDA-ARS, Sidney, MT for multiplying the entomopathogenic fungal inocula.
References
Dara, S. K. and D. Peck. 2017. Evaluating beneficial microbe-based products for their impact on strawberry plant growth, health, and fruit yield. UC ANR eJournal Strawberries and Vegetables. https://ucanr.edu/blogs/blogcore/postdetail.cfm?postnum=25122
Dara, S. K. and D. Peck. 2018. Evaluation of additive, soil amendment, and biostimulant products in Santa Maria strawberry. CAPCA Adviser, 21(5): 44-50.
Dara, S. K., S.S.R. Dara, and S. S. Dara. 2017. Impact of entomopathogenic fungi on the growth, development, and health of cabbage growing under water stress. Amer. J. Plant Sci. 8: 1224-1233. http://file.scirp.org/pdf/AJPS_2017051714172937.pdf
Dara, S. K., S. S. Dara, S.S.R. Dara, and T. Anderson. 2016. First report of three entomopathogenic fungi offering protection against the plant pathogen, Fusarium oxysporum f.sp. vasinfectum. UC ANR eJournal Strawberries and Vegetables. https://ucanr.edu/blogs/blogcore/postdetail.cfm?postnum=22199
Koike, S. T., G. T. Browne, and T. R. Gordon. 2013. UC IPM pest management guidelines: Strawberry diseases. UC ANR Publication 3468. http://ipm.ucanr.edu/PMG/r734101511.html
Partridge, D. 2003. Macrophomina phaseolina. PP728 Pathogen Profiles, Department of Plant Pathology, North Carolina State University. https://projects.ncsu.edu/cals/course/pp728/Macrophomina/macrophominia_phaseolinia.HTM
Vasebi, Y., N. Safaie, and A. Alizadeh. 2013. Biological control of soybean charcoal root rot disease using bacterial and fungal antagonists in vitro and greenhouse condition. J. Crop Prot. 2(2): 139-150.
- Author: Surendra K. Dara
Integrated pest management, commonly referred to as IPM, is a concept of managing pests that has been in use for several decades. The definition and interpretation of IPM vary depending on the source, such as a university, institute, or a researcher, and its application varies even more widely depending on the practitioner. Here are a few examples of its definitions and interpretations:
“IPM is an ecosystem-based strategy that focuses on long-term prevention of pests or their damage through a combination of techniques such as biological control, habitat manipulation, modification of cultural practices, and use of resistant varieties. Pesticides are used only after monitoring indicates they are needed according to established guidelines, and treatments are made with the goal of removing only the target organism. Pest control materials are selected and applied in a manner that minimizes risks to human health, beneficial and nontarget organisms, and the environment.” UC IPM
“Integrated Pest Management, or IPM, is an approach to solving pest problems by applying our knowledge about pests to prevent them from damaging crops, harming animals, infesting buildings or otherwise interfering with our livelihood or enjoyment of life. IPM means responding to pest problems with the most effective, least-risk option.” IPM Institute of North America
“A well-defined Integrated Pest Management (IPM) is a program that should be based on prevention, monitoring, and control which offers the opportunity to eliminate or drastically reduce the use of pesticides, and to minimize the toxicity of and exposure to any products which are used. IPM does this by utilizing a variety of methods and techniques, including cultural, biological and structural strategies to control a multitude of pest problems.” Beyond Pesticides
“IPM is rotating chemicals from different mode of action groups.” A grower
These definitions and interpretations represent a variety of objectives and strategies for managing pests. IPM is not a principle that can/should be strictly and equally applied to every situation, but a philosophy that can guide the practitioner to use it as appropriate for the situation. For example, varieties that are resistant to arthropod pests and diseases are available for some crops, but not for others. Mating disruption with pheromones is widely practiced for certain lepidopteran and coleopteran pests, but not for several hemipteran pests. Biological control is more readily employed for greenhouse pests, but not to the same extent under field conditions. While chemical pesticides should be used as the last resort, in principle, sometimes they are the first line of defense to prevent damage to the transplants by certain pests or area-wide spread of certain endemic or invasive pests and diseases.
Crop production is an art, science, and business, and by adding environmental and social factors, IPM – an approach used in agriculture – can also be influenced by a number of factors. Each grower has their own strategy for producing crops, minimizing losses, and making a profit in a manner that is acceptable to the society, safe for the consumers, and less disruptive to the environment. In other words, “IPM is an approach to manage pests in an economically viable, socially acceptable, and environmentally safe manner”. Keeping this simple, but loaded, definition in mind and considering recent advances in crop production and protection, communication technology, and globalization of agriculture and commerce, here is the new paradigm of IPM with its management, business, and sustainability aspects.
I. Management Aspect
There are four major components in the IPM model that address the various pest management options, the knowledge and resources the grower has in order to address the pest issue, planning and organization of information to take appropriate actions, and maintaining good communication to acquire and disseminate knowledge about pests and their management.
1. Pest Management:
The concept of pest control has changed to pest management over the years knowing that a balanced approach to managing pest populations to levels that do not cause economic losses is better than eliminating for environmental and economic reasons. Although the term control is frequently used in literature and conversations, it generally refers to management. A thorough knowledge of general IPM principles and various management options for all possible pest problems is important as some are preventive and others are curative. It is also essential to understand inherent and potential interactions among these management options to achieve maximum control. The following are common control options that can be employed at different stages of crop production to prevent, reduce, or treat pest infestations. Each of them may provide only a certain level of control, but their additive effect can be significant in preventing yield losses.
a. Host plant resistance: It involves the use of pest resistant and tolerant cultivars developed through traditional breeding or genetic engineering. These cultivars possess physical, morphological, or biochemical characters that reduce the plant's attractiveness or suitability for the pest to feed, develop, or reproduce successfully. These cultivars resist or tolerate pest damage and thus reduce the yield losses.
b. Cultural control: Changing agronomic practices to avoid or reduce pest infestations and damage refers to cultural control. Adjusting planting dates can help escape pest occurrence or avoid most vulnerable stages. Modifying irrigation practices, fertilizer program, plant or row spacing, and other agronomic practices can create conditions that are less suitable for the pest. Destroying crop residue and thorough cultivation will eliminate breeding sites and control soil-inhabiting stages of the pest. Crop rotation with non-host or tolerant crops will break the pest cycles and reduce their buildup year after year. Choosing clean seed and plant material will avoid the chances of introducing pests right from the beginning of the crop production. Sanitation practices to remove infected/infested plant material, regular cleaning field equipment, avoiding accidental contamination of healthy fields through human activity are also important to prevent the pest spread. Intercropping of non-host plants or those that deter pests or using trap crops to divert pests away from the main crop are some of the other cultural control strategies.
c. Biological control: Natural enemies such as spiders, predators, and parasitic wasps can be very effective in causing significant reductions in pest populations in certain circumstances. Periodical releases of commercially available natural enemies or conserving natural enemy populations by providing refuges or avoiding practices that harm them are some of the common practices to control endemic pests. To address invasive pest issues, classical biological control approach is typically employed where natural enemies from the native region of the invasive pest are imported, multiplied, and released in the new habitat of the pest. The release of irradiated, sterile insects is another biological control technique that is successfully used against a number of pests.
d. Behavioral control: Behavior of the pest can be exploited for its control through baits, traps, and mating disruption techniques. Baits containing poisonous material will attract and kill the pests when distributed in the field or placed in traps. Pests are attracted to certain colors, lights, odors of attractants or pheromones. Devices that use one or more of these can be used to attract, trap or kill pests. Pheromone lures confuse adult insects and disrupt their mating potential, and thus reduce their offspring.
e. Physical or mechanical control: This approach refers to the use of a variety of physical or mechanical techniques for pest exclusion, trapping (in some cases similar to the behavioral control), removal, or destruction. Pest exclusion with netting, handpicking or vacuuming to remove pests, mechanical tools for weed control, traps for rodent pests, modifying environmental conditions such as heat or humidity in greenhouses, steam sterilization or solarization, visual or physical bird deterrents such as reflective material or sonic devices are some examples for physical or mechanical control.
f. Microbial control: Using entomopathogenic bacteria, fungi, microsporidia, nematodes, and viruses, and fermentation byproducts of microbes against arthropod pests, fungi against plant parasitic nematodes, and bacterial and fungal antagonizers of plant pathogens generally come under microbial control.
g. Chemical control: Chemical control typically refers to the use of synthetic chemical pesticides, but to be technically accurate, it should include synthetic chemicals as well as chemicals of microbial or botanical origin. Although botanical extracts such as azadirachtin and pyrethrins, and microbe-derived toxic metabolites such as avermectin and spinosad are regarded as biologicals, they are still chemical molecules, similar to synthetic chemicals, and possess many of the human and environmental safety risks as chemical pesticides. Chemical pesticides are categorized into different groups based on their mode of action and rotating chemicals from different groups is recommended to reduce the risk of resistance development. Government regulations restrict the time and amount of certain chemical pesticides and help mitigate the associated risks.
The new RNAi (ribonucleic acid interference) technology where double-stranded RNA is applied to silence specific genes in the target insect is considered as a biopesticide application. Certain biostimulants based on minerals, microbes, plant extracts, seaweed or algae impart induced systemic resistance to pests and diseases, but are applied as amendments without any claims for pest or disease control. These new products or technologies can fall into one or more abovementioned categories.
As required by the crop and pest situation, one or more of these control options can be used throughout the production period for effective pest management. When used effectively, non-chemical control options delay, reduce, or eliminate the use of chemical pesticides.
2. Knowledge and Resources:
The knowledge of various control options, pest biology and damage potential, and suitability of available resources enables the grower to make a decision appropriate for their situation.
a. Pest: Identification of the pest, understanding its biology and seasonal population trends, damaging life stages and their habitats, nature of damage and its economic significance, vulnerability of each life for one or more control options, host preference and alternate hosts, and all the related information is critical for identifying an effective control strategy.
b. Available control options: Since not all control options can be used against every pest, the grower has to choose the ones that are ideal for the situation. For example, systemic insecticides are more effective against pests that mine or bore into the plant tissue. Pests that follow a particular seasonal pattern can be controlled by adjusting planting dates. Commercially available natural enemies can be released against some, while mating disruption works well against others. Entomopathogenic nematodes can be used against certain soil pests, bacteria and viruses against pests with chewing mouthparts such as lepidoptera and coleopteran, and fungi against sucking pests.
c. Tools and technology: A particular pest can be controlled by certain options, but they may not all be available in a particular place, for a particular crop, or within the available financial means. For example, the release of natural enemies may be possible in high-value speciality crops, but not in large acreage field crops. A particular pesticide might be registered against a pest on some crops, but not on all. Use of netting or tractor-mounted vacuums can be effective, but very expensive limiting their availability to those who can afford.
This is a critical component where diagnostic and preventive or curative decisions are made based on available and affordable control options.
3. Planning and Organization:
This component deals with the management aspect of the of the new IPM model for data collection, organization, and actual actions against pest infestations.
a. Pest monitoring: Regularly monitoring the fields for pest infestation and spread is a basic step in crop protection. Early detection in many cases can help address the pest situation by low-cost spot treatment or removal of pests or infected/infested plant material. When pest infestations continue to grow, regular monitoring is necessary to assess the damage and determine the time to initiate farm-wide control. Monitoring is also important to avoid calendar-based pesticide applications especially at lower pest populations that do not warrant treatments.
b. Managing information: A good recordkeeping about pests, their damage, effective treatments, seasonal fluctuations, interactions with environmental factors, irrigation practices, plant nutrition, and all related information from year to year will build the institutional knowledge and prepares the grower to take preventive or curative actions.
c. Corrective actions: Taking timely action is probably the most important aspect of IPM. Even with all the knowledge about the pest and availability of resources for its effective management, losses can be prevented only when corrective actions are taken at the right time. Good farm management will allow the grower to take timely actions. These actions are not only necessary to prevent damage on a particular farm, but also to prevent the spread to neighboring farms. When pest management is neglected, it leads to area-wide problems with larger regulatory, social, and economic implications.
4. Communication:
Good communication to transfer the individual or collective knowledge for the benefit of everyone is the last component of the new IPM model. Modern and traditional communication tools can be used for outreach as university and private researchers develop information about endemic and invasive pests, emerging threats, and new control strategies.
a. Staying informed: Growers and pest control professionals should stay informed about existing and emerging pests and their management options. Science-based information can be obtained by attending extension meetings, webinars, or workshops, reading newsletter, trade, extension, or scientific journal articles, and keeping in touch with researchers and other professionals through various communication channels. Well-informed growers can be well prepared to address pest issues.
b. Communication within the group: Educating farm crew through periodical training or communication will help with all aspects of pest management, proper pesticide handling, ensuring worker safety, and preventing environmental contamination. Knowledgeable field crew will be beneficial for effective implementation of pest management strategies.
c. Communication among growers: Although certain crop production and protection strategies are considered proprietary information, pests do not have boundaries and can spread to multiple fields when they are not effectively managed throughout the region. Sharing knowledge and resources with each other will improve pest control efficacy and benefit the entire grower community.
In addition to these four components with an IPM model, factors that influence profitable, safe, and affordable food production at a larger scale and their implications for global food security should also be included. There are two layers surrounding these four components addressing the business and sustainable aspects of food production.
II. Business Aspect:
Consumers want nutritious, healthy, and tasty produce that is free of pest damage at affordable prices. Growers try to meet this demand by producing food that meets all the consumer needs, while maintaining environmental and human safety and still being able to make a profit. Sellers evaluate the market demand and strategize their sales to satisfy consumers while making their own profit to stay in the business. In an ideal system, consumer, producer, and seller would maintain a harmonious balance of food production and sale. In such a system, food is safe and affordable to everyone, there will be food security all over the world, and both growers and sellers make a good profit with no or minimal risk to the environment in the process of food production. However, this balance is frequently disrupted due to i) consumers' misunderstanding of various food production systems, their demand for perfectly shaped fruits and vegetables at affordable prices or their willingness to pay a premium price for food items that are perceived to be safe, ii) growers trying to find economical ways of producing high quality food while facing with continuous pest problems and other challenges, and iii) sellers trying to market organic food at a higher price as a safer alternative to conventionally produced food. If growers implement good IPM strategies to produce safe food and consumers are aware of this practice and gain confidence in food produced in an IPM system, then sellers would be able to market what informed-consumers demand.
III. Sustainability Aspect:
As mentioned earlier, IPM is an approach to ensure economic viability at both consumer and producer level (seller is always expected to make a profit), environmental safety through a balanced use of all available pest control options, and social acceptability as food is safe and affordable.
While organic food production is generally perceived as safe and sustainable, the following examples can explain why it is not necessarily true. Organic food production is not pesticide-free and some of the pesticides used in an organic system are as harmful to humans and non-target organisms as some chemical pesticides. Certain organically accepted pesticides have toxins or natural chemical molecules that are very similar to those in synthetic pesticides. In fact, some synthetic pesticides are manufactured imitating the pesticidal molecules of natural origin. Mechanical pest control practices such as vacuuming or tilling utilize fossil fuels and indirectly have a negative impact on the environment. For example, diesel-powered tractors are operated for vacuuming western tarnished bug in strawberry 2-3 times or more each week while a pesticide application typically requires the use of tractor once every 7-14 days. To control certain pests, multiple applications of organic pesticides might be necessary with associated costs and risks, while similar pest populations could be controlled by fewer chemical pesticide applications. It is very difficult to manage certain plant diseases and arthropod pests through non-chemical means and inadequate control not only leads to crop losses, but can result in their spread to larger areas making their control even more difficult. Many growers prefer a good IPM-based production to an organic production for the ease of operation and profitability. However, they continue to produce organic food to stay in business.
While middle and upper-class consumers may be willing to pay higher prices for organically produced food, many of the low-income groups in developed and underdeveloped countries cannot afford such food. Organic food production can lead to social inequality and a false sense of wellbeing for those can afford. Food security for the growing world population is necessary through optimizing input costs, minimizing wastage, grower adoption of safe and sustainable practices, and consumer confidence in food produced through such practices. IPM addresses all the economic, environmental, and social aspects and provides safe and affordable food to the consumers and profits to producers and sellers, while maintaining environmental health.
- Author: Surendra K. Dara
- Author: Dave Peck, Manzanita Berry Farms
In a continuous effort to explore the potential of additive, soil amendment, biostimulant, and other products, a new study was conducted in a conventional strawberry field at the Manzanita Berry Farms in Santa Maria. The following treatments were administered at different times, from planting till the end of production season, as requested by the manufacturer.
- Untreated control: Other than the soil incorporated fertilizers during the field preparation, no other nutrient inputs were added during the study.
- Grower standard: Transplants were dipped in Switch 62.5WG (cyprodinil+fludioxonil, at 5 oz/100 gal) before planting and a proprietary nutrient regimen that included administration of a humic acid-based product was followed.
- Innovak Global regimen: Nutrisorb-L (a blend of polyhydroxy carboxylic acids) at 28 fl oz/ac, starting 2 wk after planting and every 3 wk thereafter through drip. Packhard (carboxylic acids with calcium and boron) at 28 fl oz/ac, starting at the first fruit set (early January) and every 2 wk thereafter as a foliar spray.
- TerraVesco regimen: A microbe-rich Vermi-extract (worm extract) at 10% vol/vol as a transplant dip for 3 hours, followed by application through drip at 7.5 gal/ac after planting, and again in December, 2017 and January, 2018.
- Fertum regimen: Transplant dip in 1% vol/vol of Germinal Plus (a product from marine algae), followed by drip applications of Booster (a biostimulant and a natural organic fertilizer made from seaweed) at 0.5 gal/ac in late November and late December, 201; Silicium PK (a biostimulant and a natural organic fertilizer based on silicon enriched with phosphorus, potassium and seaweed extracts) at 0.5 gal/ac late December, 2017 and once a month starting from mid February to early July, 2018; and Foliar (a biostimulant and a natural organic fertilizer from marine algae) at 0.5 gal/ac in mid and late January.
- Shemin Garden regimen: EcoSil (a silica fertilizer) at 800 ml/ac once a month starting from early December, 2017 to May, 2018 through drip, and at 200 ml/ac in early May and June, 2018 as a foliar spray; ComCat (based on a plant extract) at 20 gr/ac and EcoFlora (a consortium of Azotobacter spp., Bacillus spp., Paenibacillus spp., Pseudomonas sp., Trichoderma spp., and Streptomyces spp.) at 12 oz/ac one week after EcoSil through drip until May, 2018 and ComCat at 10 gr/ac and EcoFlora at 12 oz/ac as a foliar spray in May and June, 2018.
- GrowCentia regimen-low: Yeti containing 1% bacterial culture (of Pseudomonas putida, Citrobacter freundii, Comamonas testosterone, and Enterobacter cloacae) and 2% alfalfa extract applied at 0.6 ml/gal through drip for 90 min weekly from the first drip application.
- GrowCentia regimen-high: Yeti at 1 ml/gal through drip for 90 min weekly from the first drip application.
- NanoChem regimen: EX10, a biodegradable fertilizer additive containing thermal polyaspartate at 1 qrt/ac through first drip after planting with follow up applications in early January (first bloom), mid February, and mid May, 2018. The active ingredient binds with cations such as ammonium, calcium, copper, iron, magnesium, manganese, potassium, and zinc and improves their availability for the plant.
- BiOWiSH regimen 1: Formula 1 at 1.33 oz/gal for transplant dip followed by 3.53 oz/ac through drip starting 2 wk after planting and every 4-5 wk thereafter.
- BiOWiSH regimen 2: Formula 1 at 1.33 oz/gal for transplant dip followed by 3.53 oz/ac as a foliar srpay starting 2 wk after planting and every 4-5 wk thereafter.
- BiOWiSH regimen 3: Formula 1 at 1.33 oz/gal for transplant dip followed by 3.53 oz/ac through drip starting 2 wk after planting alternated with a foliar spray every 4-5 wk.
- BiOWiSH regimen 4: Formula 1 at 1.33 oz/gal for transplant dip followed by BiOWiSH Crop 16-40-0, a microbial consortium (Bacillus amyloliquefaciens, B. lichenoformis, B. pumilus, and B. subtilis)at 3.53 oz/ac through drip starting 2 wk after planting and every 4-5 wk thereafter.
Each treatment contained a 165' long 5.7' wide bed and replicated four times in a randomized complete block design. A 15' long plot in the center of the bed was marked and netted for collecting yield and some other parameters that were compared. Strawberry cultivar BG 6-30214 was planted on 7 November, 2017. Other than the untreated control, all other products were administered on top of the grower standard fertility program. However, only the grower standard transplants were dipped in Switch 62.5WG before planting.
Various parameters were measured during the vegetative growth and fruit production periods to evaluate the impact of the treatments on crop growth, health, and yield. Data were analyzed using ANOVA and LSD test was used to separate significant means.
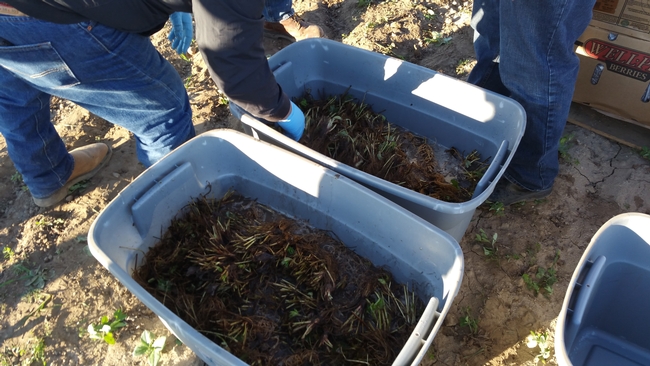
Transplant treatment (above) and drip application (below). Photos by Tamas Zold
Canopy growth: Canopy growth was observed on 11 December, 2017, 7 and 30 January, and 8 February, 2018 by measuring the size of the canopy along and across the length of the bed from 20 random plants per bed and calculating the area. Canopy size significantly (P = 0.0261) different among the treatments only on the last observation date where plants treated with EX10 and the GrowCentia product at the low concentration were larger than those in the grower standard.
Electrical conductivity and temperature of soil: From two random location on each bed, electrical conductivity (EC in dS/m) and temperature (oC) were measured about 3 inches deep from the surface on 12 and 25 January, 7 February, 19 March, 18 April, and 29 May, 2018. Only soil temperature on 25 January significantly (P = 0.0007) varied among treatments where the difference between the highest (untreated control) and the lowest (Vermi-extract) values was 0.8oC.
Dead plants: The number of dead plants represents empty spots in the bed due to the death of transplants. There were no obvious signs of disease or a particular stress factor associated with those plants except that they were randomly distributed within each bed and throughout the field. When counted on 18 April, 2018, BiOWiSH regimen 4, Fertum regimen, GrowCentia product at the high rate, and Innovak Global regimen had
Fruit diseases: Fruit harvested on 12 March, 3 and 13 April, and 17 May, 2018 from each marked plot was incubated at room temperature in dark in plastic containers and the fungal growth was rated 3 and 5 days after harvest (DAH) using a scale of 0 to 4 where 0=no fungal growth, 1=1-25%, 2=26-50%, 3=51-75%, and 4=76-100% fungal growth. Botrytis fruit rot or grey mold was predominant during the first two observation dates and the growth of other fungi (possibly Rhizopus spp.) was also seen during the last two dates. In general, fruit disease occurred at low levels throughout the observation period with
Sugar content in fruit: Sugar content was measured from two harvest-ready berries per bed on 17 May, 2018 using a handheld refractometer. Sugar content varied from 8.06 oBx (Innovak Global regimen) to 9.53 oBx (grower standard).
Fruit firmness: Fruit firmness was measured from eight randomly collected harvest-ready berries from each bed on 28 June, 2018. Firmness varied from 0.82 kgf (Fertum and Shemin Garden regimens) to 0.98 kgf (untreated control).
Fruit yield:Strawberries were harvested from 6 February to 22 June, 2018 on 36 dates. When compared to the grower standard, the marketable berry yield was 16.2, 15.1, 13.7, and 13% higher in Fertum regimen, EX10 treatment, Innovak Global regimen, and BiOWiSH regimen 4, respectively. The marketable berry yield was 9.8, 9, 7.5, and 6.8% higher in those respective treatments over the yield from untreated control.
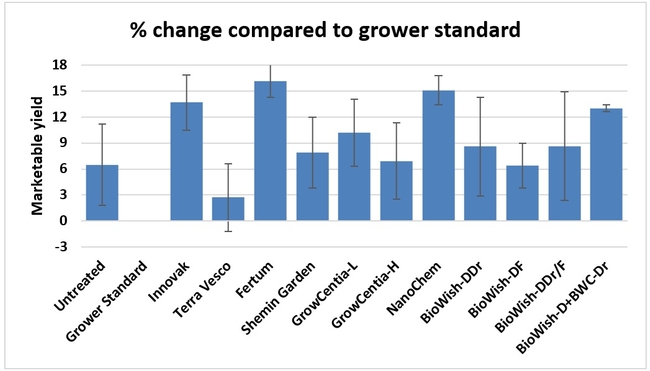
It took 23 harvest dates in three months (from February to April, 2018) to obtain the first third of the total seasonal yield while the remaining two-thirds were obtained from seven harvest dates in May and six dates in June. Marketable fruit yield was higher than the grower standard in all treatments and higher than the untreated control in most treatments.
In general, fruit yields were higher and the pest and disease pressure was lower than usual during the study period. Aleo, a garlic oil based fungicide, at lower label rates was periodically used for disease management and bug vacuums were operated a few times against the western tarnished plant bug as a standard across all treatments.
This study evaluated some treatment regimens as recommended by the collaborating manufacturers and some of them appear to have a potential for use in strawberry production. These results help the manufacturers fine tune their recommendations for achieving better yields through additional studies.
Acknowledgments: We thank the planting and harvest crew at Manzanita Berry Farms for their help with the crop production aspects, Chris Martinez, Tamas Zold, and Maria Murrietta for their technical assistance, Sumanth Dara for statistical analysis, and the support of the industry collaborators who funded the study.
- Author: Christian Nansen, Elvira de Lange, Alison Stewart, Department of Entomology and Nematology, UC Davis
- Author: Mark Edsall, California Strawberry Commission
- Author: Surendra K. Dara
The western tarnished plant bug (or lygus bug) and different species of spider mites are major arthropod pests in California strawberries. While predatory mite releases are very popular for controlling spider mites in both organic and conventional fields, a significant amount of chemical pesticides are used for arthropod pest management in conventional fields. Nearly 280,000 pounds of active ingredient of at least 30 chemical and botanical pesticides were used in 2016 in California strawberries (USDA-NASS, 2016; CDPR, 2017) and malathion, bifenazate, naled, acequinocyl, and fenpropathrin were the most common of about 79,000 pounds of chemical pesticides. A uniform and thorough coverage of pesticide sprays is essential for effective pest management and also for reducing excessive pesticide use that could lead to resistance and environmental health issues (Shi et al., 2013). Evaluation of pesticide spray applications and their performance will help improve current pest management strategies in strawberry as they did in other crops (Nansen et al., 2011; Nansen et al., 2015).
Several factors such as the tractor speed, spray nozzles, spray volume, boom height, adjuvants, pressure, canopy characters, micro and macroclimatic conditions influence the spray coverage. A better understanding of these factors will help improve the pesticide use and efficacy, optimize the cost, and reduce pesticide drift and other associated risks (Nansen and Ridsdill-Smith, 2013).
A study was conducted during 2016 and 2017 to evaluate multiple spray configurations under varying weather conditions where more than 4000 data points were collected. Data for only two spray configurations, using Albuz ATR 80 Lilac and Albuz ATR 80 Green nozzles, are shown here.
Configuration 1: Albuz ATR 80 Lilac nozzles were used in 144 experimental applications delivering 32-80 gallons of spray volume per acre. Water-sensitive spray cards (TeeJet, Wheaton, IL) were clipped to the petioles of strawberry leaves in horizontal and vertical orientation (1 card per application for each orientation). They were placed in the strawberry canopy prior to spray applications and the coverage was determined based on the pattern on the cards using the SnapCard smartphone application. Data suggested that spray volume does not always translate into a good spray coverage (Fig. 1). There was a wide variation in the coverage that ranged from 0-55% at 33 gpa and 5-80% at 80 gpa suggesting the influence of other factors. Taking the operational and weather conditions into account, a multiple linear regression analysis was conducted to measure the relationship between predicted and observed spray coverage which appeared to have a linear correlation (Adjusted R2=0.27; F+52.6; P < 0.001) (Fig. 2). Wind speed, wind gust, ambient temperature, pressure, and tractor speed were used as explanatory variables in this analysis. Based on this regression model, four possible scenarios were developed with predicted spray coverage values (Table 1). A 2 mph increase in the wind speed from 6 mph to 8 mph could reduce the spray coverage from 61 to 45% when the tractor runs at 1 mph or from 30 to 13% when the tractor runs at 2 mph.
Configuration 2: Albuz ATR 80 Green nozzles were used in 276 experimental applications delivering 180-440 gallons of volume per acre. Since the droplet size from the Green nozzle is much larger than that from the Lilac nozzle, a better coverage is expected with the presumption of lesser sensitivity to environmental and operating conditions. However, data from the spray cards indicated a poor relationship between the spray volume and coverage under this configuration as well (Fig. 3). For example, both 200 and 350 gpa had a similar spray coverage. Multiple linear regression analysis, using strawberry canopy characteristics (plant height and width, dry weight, and canopy coverage), operating and weather conditions as explanatory variables, showed a significant linear correlation (Adjusted R2=0.45; F=199.5; P < 0.001) between observed and predicted spray coverages (Fig. 4). A prediction model under this configuration showed nearly 20% decline in spray coverage when the tractor speed increased from 1 to 2 mph (Table 2). Wind speed appeared to have a minimal impact, probably due to the droplet size.
This study demonstrates the importance of weather and operating conditions on spray coverage. Additional data will be collected in 2018 to expand our understanding of the factors that influence spray coverage. These studies will be useful to determine appropriate operating conditions such as the spray volume, tractor speed, and types of nozzles and identify weather conditions that are ideal to achieve good coverage. A free smartphone application is under development for the growers and PCAs to input weather and operating conditions to predict the spray coverage. This information will ultimately improve the pest control efficacy and contribute to sustainable pest management practices.
Acknowledgments: We thank the financial support of the California Strawberry Commission and the collaboration of several growers. We also thank the technical assistance of Daniel Olivier, Marianna Castiaux, and Ariel Zajdband, California Strawberry Commission and Robert Starnes, Jessie Liu, Laurie Casebier, Haleh Khodaverdi, and Isaac Corral, UC Davis.
References
CDPR. 2017. Summary of pesticide use report data 2016: California Department of Pesticide Regulation, p. 909.
Nansen C, Ferguson JC, Moore J, Groves L, Emery R, Garel N and Hewitt A. 2015. Optimizing pesticide spray coverage using a novel web and smartphone tool, SnapCard. Agronomy for Sustainable Development: 1-11. DOI: 10.1007/s13593-015-0309-y.
Nansen C & Ridsdill-Smith TJ (2013) The performance of insecticides – a critical review: Insecticides (ed. by S Trdan) InTech Europe, Croatia, pp. 195-232.
Nansen C, Vaughn K, Xue Y, Rush C, Workneh F, Goolsby J, Troxclair N, Anciso J, Gregory A, Holman D, Hammond A, Mirkov E, Tantravahi P and Martini X (2011) A decision-support tool to predict spray deposition of insecticides in commercial potato fields and its implications for their performance. Journal of Economic Entomology 104: 1138-1145. DOI: 10.1603/EC10452.
Shi M, Collins PJ, Ridsdill-Smith TJ, Emery RN and Renton M. 2013. Dosage consistency is the key factor in avoiding evolution of resistance to phosphine and population increase in stored-grain pests. Pest Management Science 69: 1049–1060. DOI: 10.1002/ps.3457.
USDA_NASS. 2016. Quick stats.